Table of Contents
Abstract 4
1. Magnetic
Materials 5
1.1 Magnetic
Susceptibility 5
1.2 Magnetic
Permeability 6
1.3
Magnetostriction 6
2. Laser 6
2.1 Operation of a
Laser 7
2.2 Principle of
Laser 7
2.3 Properties of
Laser 8
2.4 Types of
Laser 8
2.5 Power of
Laser 9
2.6 Applications of
Laser 10
2.6.1 Data
Storage 10
2.6.2 Laser
Cutting/Welding 10
2.6.3
Optical Fiber
Communication
Systems 11
2.6.4 Laser
Printing 11
2.6.5 Medical
Applications 11
3. Types of Magnetic
Materials
and
Laser 12
3.1 Diamagnetic
Materials 12
3.1.1
Real-world application of
diamagnetic materials 13
3.1.2
Laser and
diamagnetic
materials 13
3.1.3
Further
Research 13
3.2
Paramagnetic
Materials 14
3.2.1
Real-world
application of
paramagnetic materials 15
3.2.2
Laser and
paramagnetic
materials 15
3.2.3
Further
Research 15
3.3
Ferromagnetic
Materials 16
3.3.1
Real-world
application of
ferromagnetic materials 17
3.3.2
Laser and
ferromagnetic
materials 18
3.3.3
Further
Research 18
3.4
Ferrimagnetic
Materials 19
3.4.1
Real-world
application of
ferrimagnetic materials 20
3.4.2
Laser and
ferrimagnetic
materials 20
3.4.3
Further
Research 20
3.5
Antiferromagnetic
Materials 21
3.5.1
Real-world
application of
antiferromagnetic materials 22
3.5.2
Laser and
antiferromagnetic
materials 22
3.5.3
Further
Research 23
Results 23
References 24
Abstract
This study aspires to discuss the connection between laser and
magnetic
materials. It outlines how laser technology has enabled the development of magnetic materials
with
superior properties, such as high coercivity, high saturation, and low magnetostriction.
Laser-based
deposition of magnetic layers has also enabled the fabrication of magnetic nanostructures and
microstructures. The study also examines the potential applications of laser-treated magnetic
materials
in various fields, such as power electronics, data storage, and medical imaging.
While the author wants to illustrate how laser energy can be used to
create
magnetization and how the strength of that magnetization can be altered depending on the laser
beam’s power and wavelength, the author cannot conclude due to technological constraints.
Additionally, how the combination of laser and magnetic materials can be used to create magnetic
fields
and how laser-induced changes in magnetic materials can improve the functionality of devices
such as
sensors and actuators. Ultimately, through this study, the author wants to provide fundamental
insight
into the potential applications of laser technology when combined with different magnetic
materials.
The study concludes that laser technology provides a powerful tool
for
developing advanced magnetic materials with improved performance.
Keywords: Magnetic Materials, Effect
of
magnetic materials on laser, laser, Magnetization and lasers, Energy, Effect of laser on
magnetic
fields
1. Magnetic Materials
The substances that can be magnetized or induced to possess magnetic properties
are
known as magnetic materials. These materials are characterized by their ability to interact with
an
external magnetic field, allowing them to be attracted to or repelled by particular objects. The
magnetic properties of materials depend on their composition and the arrangement of their
atoms.
The phenomenon of magnetism is based on the behavior of electrons,
particles
found in atoms. Electrons are components of electric current and possess a property known as
spin. This
property causes the electrons to act as tiny bar magnets, and each electron is attracted or
repelled by
the external magnetic field. When a material is placed in a magnetic field, the electrons within
the
material align themselves in the direction of the external field. This alignment of electrons is
referred to as magnetization, the source of the material's magnetic properties.
The properties of magnetic materials, such as their magnetic
susceptibility,
permeability, and magnetostriction, can be used to determine their suitability for different
applications. For example, magnetic materials are used in various electronics, automotive, and
aerospace
industries to produce components such as motors and actuators. Magnetic materials are also used
in
medical devices and magnetic resonance imaging (MRI) scanners.
1.1 Magnetic Susceptibility
The strength of a material's magnetic properties is determined by
its
magnetic susceptibility, which measures how easily the external field can align the electrons.
Magnetic
susceptibility is determined by the material's composition, structure, and arrangement of
its atoms.
The susceptibility of a material can be expressed mathematically by the equation:
χ = Σμ/H
where μ is the magnetic moment of an
electron,
H is the external field, and χ is the material’s magnetic
susceptibility.
1.2 Magnetic Permeability
The magnetic behavior of a material is also determined by its
magnetic
permeability, which measures how much magnetic flux is generated when placed in a magnetic
field. The
permeability of a material can be expressed mathematically by the equation:
μ = μ0(1 +
χ)
where μ is the magnetic permeability,
μ0 is the permeability of a
vacuum,
and χ is the material’s magnetic
susceptibility.
1.3 Magnetostriction
The magnetic behavior of a material is also affected by its
magnetostriction,
which measures how much strain is generated in a material when placed in a magnetic field. The
magnetostriction of a material can be expressed mathematically by the equation:
ε = ε0(1 +
λ)
where ε is the strain,
ε0 is the strain of a
vacuum, and
λ is the material’s
magnetostriction.
2. Laser
LASER, or Light Amplification through Stimulated Emission of
Radiation, is a
light source capable of producing powerful, highly directional light beams. Lasers generate and
emit
coherent light, usually in the form of an intense, narrow beam. Lasers are widely used in many
scientific, industrial, and medical applications, including optical communication, remote
sensing,
materials processing, and spectroscopy. Let us discuss the properties and characteristics of
laser
light, the physics behind lasers, the equations governing laser behavior, the properties of
lasers, and
the various types of lasers.
Laser light is a type of electromagnetic radiation, meaning it is
made up of
a combination of electric and magnetic fields. Light with a single wavelength and phase, such as
that
produced by a laser, is known as coherent light. This is in contrast to incoherent light, which
has
multiple wavelengths and phases. Laser light is highly directional and can be focused into a
highly
intense beam.
2.1 Operation of a Laser
The operation of a laser begins with the population inversion of an
atomic
medium. This occurs when more atoms or molecules are in an excited state than in the ground
state. A
pump source is then used to induce excitation in the medium. This pump source can be either
electrical
or optical. Once the population inversion has been achieved, the atoms or molecules emit photons
when
they transition to their ground state. The emitted photons will then interact with other excited
atoms
or molecules, stimulating emission. This process continues until all the excited atoms or
molecules have
returned to their ground states and a beam of laser light is produced.
2.2 Principle of Laser
A laser is based on the principle of stimulated emission, which
states that
when a photon of light stimulates an excited atom or molecule, it can cause the emission of
another
photon with identical properties. Stimulated emission is the basis for laser operation. The
photons
emitted from the excited state are referred to as 'laser light.'. In this process,
electrons in
an atom or molecule are stimulated by an external energy source, such as an electric current, to
move
from higher energy levels to lower energy levels. As the electrons drop to lower energy levels,
they
emit a photon of light. Einstein's equation describes this process for the stimulated
emission of
radiation:
E = hf
where E is
the photon's energy, h is Planck’s constant,
and
f is the frequency of the light emitted.
This process is repeated in a laser as the photons produced stimulate
other
electrons to drop to lower energy levels and emit additional photons. This creates a chain
reaction, or
cascade, of stimulated emissions, allowing a single photon to produce many more. The intensity
of the
laser beam is determined by the number of photons produced in this cascade.
2.3 Properties of Laser
The properties of laser light depend on several factors, including
the type
of gain medium used, the excitation mechanism, and the resonator design. Laser light usually has
a
narrow spectral bandwidth, meaning only light with a specific frequency is generated. In
addition, laser
light is coherent, which means that all the waves have the same frequency and phase.
Furthermore, laser
light has a high degree of directionality and can be focused on small spots.
2.4 Types of Laser
There are various types of lasers available for different
applications.
These include gas lasers such as
- helium-neon lasers (HeNe)
- carbon dioxide lasers (CO2)
- solid-state lasers such as Nd: YAG (neodymium-doped
yttrium
aluminum garnet) and ruby lasers
- semiconductor lasers such as diode lasers
- other types, such as excimer and free-electron
lasers
Each type has its advantages and disadvantages, depending on its
application.
2.5 Power of Laser
Laser is based on the principle of stimulated emission and is
governed by
complex equations. Understanding these equations is essential for successfully designing and
operating
laser systems. The power output of a laser can be determined using the following equation:
P = hν ∙ η ∙ Φ
where P is the power output (W), h is Planck’s
constant
(6.62607015 x 10-34 Js), ν is the frequency of the laser light (Hz), η is the efficiency of the laser (%),
and Φ is the number of photons
per second ( s-1 ).
The equations governing the behavior of a laser are complex and
involve many
variables. The most important of these is the gain coefficient, which describes the stimulated
emission
rate in the laser cavity. The equation gives the gain coefficient:
G = σ(n2 -
n1)
where G is the gain coefficient,
σ is the stimulated emission cross-section, and
n1 and n2 are the populations of
atoms in the upper and lower energy
levels, respectively.
2.6 Applications of
Laser
Lasers are powerful and versatile light sources with many
applications.
Lasers generate intense beams of coherent light through stimulated emission. They are used for
many
applications due to their unique properties, such as narrow spectral bandwidth, coherence,
directionality, and focusing capability. Depending on the application, several different types
are
available, and their power output can be determined using an equation derived from Planck's
constant. There are multiple applications of LASER.
2.6.1 Data
Storage
Lasers are used to read and write data on CDs, DVDs, and other media
storage
devices.
The laser reads the information from a disk by shining light onto its surface and reflecting
it
off of
various layers in the disk. It can then detect changes in reflected light intensity
representing
stored
data bits (formula: n = 2n where n is
the
number of
bits). Let us get into its details. Data storage involves storing files digitally on discs
such
as CDs
and DVDs; these discs contain tiny bumps inside them, which act as 0s and 1s representing
binary
code
depending upon their presence or absence, respectively. A laser beam shines onto these bumps
producing
different intensities depending upon their presence, i.e., whether they reflect any signal,
forming
readable chunks representing the entire file. Once formed, this file can be accessed anytime
just by
rereading these tiny pits with the help of the same technology.
2.6.2 Laser
Cutting/Welding
Lasers are commonly used for cutting or welding materials such as metal or
plastic
using a
process called "heat conduction," which uses heat generated by the laser beam to
melt
through
the material (formula: Heat = Power x Time).
This
process reduces waste compared to traditional cutting methods, allowing finer cuts with more
precise
control over the material shape.
2.6.3 Optical
Fiber
Communication
Systems
Lasers are employed in fiber optic communication systems that use
optical fibers
instead of electrical wires to transmit signals between locations. These light pulses
carrying
digital
information travel down these cables at incredibly high speeds, making them an efficient way
to
quickly
send large amounts of data over long distances without interference from external sources
like
radio
waves or electric fields. Formula:
c = f λ
where c is the speed of light,
f is frequency, and λ
is
the wavelength.
2.6.4 Laser
Printing
Laser printing involves transferring images onto paper. The printer
contains a
photoconductor drum that stores electrons when exposed to light and transfers those
electrons
onto paper
when heated up, causing toner particles attached to the paper fibers to create text or
graphics
on pages
printed from the device. This method provides higher-resolution prints than inkjet printers,
with
sharper details and deeper colors due to increased accuracy during transfer
processes.
2.6.5 Medical
Applications
Lasers have many medical applications, including vision correction surgery,
also
known as
LASIK, which reshapes corneal tissue so that it better focuses incoming light into one
single
focal
point on the retina (formula: P = F / D where
P is power density, F is
total
energy outputted per unit time and D stands
for
distance) This procedure has been immensely successful since its introduction due firstly
because this
technique requires no incision &damage caused during surgery could not be reversed if
something went
wrong secondly results were predictable & accurate thus becoming popular among people
who
wanted
improved vision without undergoing risky surgeries like Radial keratotomy.
3. Types of Magnetic Materials and
Laser
Magnetic materials can be broadly classified into five categories:
diamagnetic, paramagnetic, ferromagnetic, ferrimagnetic, and antiferromagnetic. Each category
has unique
characteristics and behavior and is used in various applications.
3.1 Diamagnetic Materials
Diamagnetic materials are materials that are
weakly
repelled by magnetic fields. This means that when a diamagnetic material is placed in a magnetic
field,
it will tend to move away from the field. The magnetic response of diamagnetic materials is
typically
minimal and not strongly affected by external magnetic fields. Examples of diamagnetic materials
include
copper, aluminum, and gold.
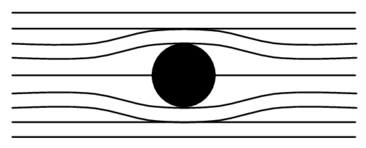
Figure: Magnetic Field and Diamagnetic Materials
One of the critical properties of diamagnetic materials is their
susceptibility, which measures their response to an applied magnetic field.
The susceptibility of a diamagnetic material is typically minimal and
is
given by the formula:
χ = -μ0 / 4π * M
where μ0 is
the
magnetic permeability of vacuum, and M is
the
magnetization of the material. The negative sign indicates that the magnetic moment of a
diamagnetic
material is opposite in direction to the applied magnetic field.
3.1.1
Real-world
application of diamagnetic
materials
A real-world application of diamagnetic materials uses levitation
technology.
Because magnetic fields weakly repel diamagnetic materials, they can be suspended in mid-air
using a
strong magnetic field. This technology is used in various applications, such as designing
magnetic
levitation trains and studying quantum phenomena.
3.1.2 Laser and
diamagnetic
materials
Laser light can be used to manipulate diamagnetic materials.
Diamagnetic
materials are weak and negatively susceptible to magnetic fields, meaning they are repelled
by
the
field. When laser light is shone on a diamagnetic material, it interacts with its electrons,
causing
them to vibrate. This vibration creates an opposing magnetic field, pushing the material
away
from the
laser source. This property can be utilized in various applications such as levitation,
transport, and
manipulation of materials.
3.1.3 Further
Research
Further research in the field of diamagnetic materials could
focus on
developing new materials with enhanced diamagnetic properties and exploring new applications
for
diamagnetism, such as in the design of magnetic levitation systems for transportation and
other
purposes.
3.2 Paramagnetic Materials
Paramagnetic materials are materials
that are
weakly attracted to magnetic fields. This means that when a paramagnetic material is placed in a
magnetic field, it will tend to move toward the field. The magnetic response of paramagnetic
materials
is typically tiny but much larger than that of diamagnetic materials. Examples of paramagnetic
materials
include iron, cobalt, and nickel.
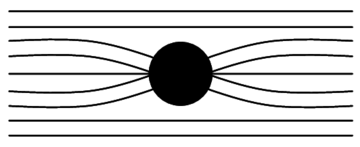
Figure: Magnetic Field and Paramagnetic Materials
The formula for the magnetic susceptibility of paramagnetic materials
is:
χ = μ0 /
4π *
M
where μ0 is
the
magnetic permeability of vacuum, and M is
the
material’s magnetization. The positive sign indicates that the magnetic moment of a
paramagnetic
material is in the same direction as the applied magnetic field.
Paramagnetic materials do not show any significant amount of
spontaneous
polarization. However, when exposed to specific external electromagnetic fields, they become
somewhat
polarized, resulting from aligning electron orbitals parallel or antiparallel resulting from the
spin-orbit coupling. Paramagents exhibit a high degree of susceptibility. Their moments increase
exponentially when exposed under certain conditions, as shown via the equation:
X = C + BH2 +
DH4 + FH6 …etc…
here, X represents susceptibility while
BH stands for the linear response. In contrast,
DH & FH stand for
nonlinear
responses usually found at lower temps indicating temperature-dependent behavior.
3.2.1 Real-world
application
of
paramagnetic
materials
One real-world application of paramagnetic materials is magnetic
resonance
imaging (MRI) in medicine. Because paramagnetic materials are weakly attracted to magnetic
fields, they
can be used to create strong magnetic fields that can be used to study the human body. This
technology
is used in various medical applications, such as diagnosing diseases and injuries.
3.2.2 Laser and
paramagnetic
materials
Paramagnetic materials contain
unpaired
electrons, and when exposed to laser light, these materials become more paramagnetic,
allowing
them to
be manipulated via the laser's magnetic field. This makes laser manipulation of
paramagnetic
materials possible, allowing for a range of applications, including targeted drug delivery
and
tissue
engineering. By controlling the laser's intensity, frequency, and pulse width, it is
possible to
create precise and accurate manipulation of paramagnetic materials.
3.2.3 Further
Research
Further research in the field of paramagnetic materials could
focus
on
developing new materials with enhanced paramagnetic properties, as well as on the
exploration of
new
applications for paramagnetism, such as in the design of magnetic resonance imaging systems
for
medical
and other purposes.
3.3 Ferromagnetic Materials
Ferromagnetic materials are characterized by
their
ability to be magnetized and retain their magnetization even without an external magnetic field.
Examples of ferromagnetic materials include iron, cobalt, and nickel. These materials exhibit
strong
magnetic properties, with their magnetic moments aligned in the same direction within the
material. The
magnetic response of ferromagnetic materials is typically much larger than that of paramagnetic
materials, and they can produce their magnetic fields.
The magnetic behavior of ferromagnetic materials can be described by
the
Curie-Weiss law, given by:
M(T) = C / (T - Θ)
where M is the magnetization, T is the temperature, C is the
Curie
constant, and Θ is the Curie
temperature.
Ferromagnetic materials have their magnetic memory or hysteresis,
which is
the material's ability to retain its magnetization without an external magnetic field. This
results
from the alignment of the magnetic moments of the atoms within the material. The magnetic moment
of an
atom is a measure of its magnetic properties and is related to its angular momentum and spin. In
ferromagnetic materials, the magnetic moments of the atoms are aligned in the same direction,
resulting
in a strong overall magnetic field.
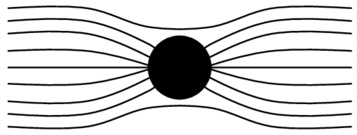
Figure: Magnetic field and Ferromagnetic Materials
Ferromagnets typically contain iron (Fe) but may also contain cobalt (Co),
nickel (Ni),
or some alloys containing these elements, like Alnico, which contains Aluminium(Al), Nickel(Ni),
and
Cobalt(Co). These elements form domains within the ferromagnet, where each domain acts like a
tiny bar
magnet with its north and south pole. If enough domains line up in one direction, then they
combine,
creating an overall macroscopic magnetic moment known as spontaneous magnetization, Ms defined by the equation
below:
Ms =
NAμ
where μ is the Bohr Magneton given by the equation:
μ = 927400 Am2/kg
Furthermore, NA is Avogadro's number given by the
equation:
NA =
6.02214076*1023 molecules/mol
3.3.1 Real-world
application of
ferromagnetic
materials
One real-world application of ferromagnetic materials is
electromagnets. In
this application, ferromagnetic materials create strong magnetic fields that can be turned
on
and off by
applying an electric current. This allows for the creation of loudspeakers and
electromechanical
devices, such as electric motors and generators. In these applications, the magnetization of
ferromagnetic materials generates magnetic fields that can convert electrical energy into
mechanical
motion and vice versa.
Because ferromagnetic materials can be magnetized and retain
their
magnetization, they can store digital information in magnetic patterns. This technology is
used
in
various applications, such as computers and other electronic devices. Ferromagnets are also
used
in
magnetic storage devices, such as hard drives and tapes.
Overall, ferromagnetic materials are an important class of
materials
with
various applications in various fields.
3.3.2 Laser
and
ferromagnetic
materials
Laser-ferromagnetic materials combine the properties of lasers
and
ferromagnetic materials to create a powerful, energy-efficient, and versatile new
technology.
Laser-ferromagnetic materials are characterized by their ability to convert laser energy
into
electrical
energy via the magnetization of the ferromagnetic material. This conversion process is
called
the
Magneto-Optical Kerr Effect (MOKE) and is based on the interaction between laser light and
the
atomic
structure of ferromagnetic materials. This effect enables the creation of novel devices such
as
magnetic
field sensors, magneto-optical switches, and magnetic recording media.
3.3.3 Further
Research
Laser-ferromagnetic material interactions are a rapidly
developing
field of
research due to the potential applications of these materials. The development of these
applications is
expected to revolutionize how we interact with our environment in the near future. Further
research in
this field has the potential to unlock new technologies and improve existing ones. It could
focus on
developing new materials with enhanced ferromagnetic properties and exploring new
applications
for
ferromagnetism, such as in the design of magnetic storage devices with higher capacity and
improved
performance. Potential applications in spintronic devices and quantum computing can also be
explored.
3.4 Ferrimagnetic Materials
Ferrimagnetic materials are magnetic
materials in
which the magnetic moments of the atoms are aligned in opposite directions, leading to the
presence of
two magnetic sublattices. This results in the material having a net magnetization but a reduced
magnetic
moment compared to a ferromagnetic material. The Brillouin function can mathematically describe
this
reduced magnetization by
B(T) = coth(J / T) - 1 / J / T
where J is the exchange interaction
between the
magnetic sublattices, and T is the
temperature.
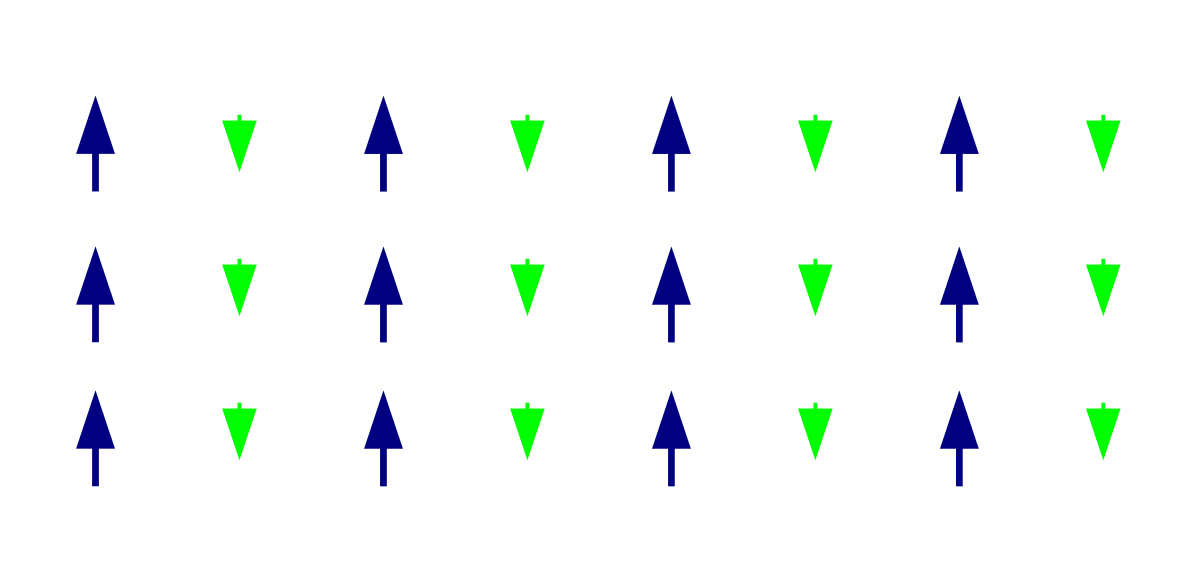
Figure: Magnetic Moments of Paramagnetic Materials
When Ferrimagnets are aligned in one direction, a net dipolar moment exists
between the
two unlike atoms resulting from their competing moments, causing them not entirely to cancel
out,
creating an induced moment Mi. The equilibrium state
between this
induced moment and interaction energy causes an exchange bias force EB which can be described
using the
equation below:
EB = −2KV +
2JMi2
where K represents
uniaxial anisotropy constant, J represents exchange
coupling
constant V, volume per unit cell respectively. As
K and J get
intense, more energy
must be supplied to make changes in alignment, thus making stronger magnets with higher coercive
fields.
3.4.1 Real-world
application of
ferrimagnetic
materials
One real-world application of ferrimagnetic materials is in
magnetic
data
storage. In this application, the reduced magnetization of ferrimagnetic materials allows
for
higher
data density on a magnetic storage medium, such as a hard disk drive.
3.4.2 Laser and
ferrimagnetic
materials
The relationship between laser
and
ferrimagnetic materials is based on the fact that laser light can induce magnetization in
ferrimagnetic
materials. The light excites electrons in the material, causing them to move and interact
with
the
material’s magnetic dipole moments. This interaction creates a magnetic field, which
induces a
magnetic moment in the material, allowing it to become magnetized. This process is known as
optical
switching, and it has been used to create various applications such as magnetic memory, data
storage,
and spintronic devices. Laser light can also be used to control the properties of
ferrimagnetic
materials by manipulating their magnetic fields and orientations.
3.4.3 Further
Research
There is still much to be explored in ferrimagnetic materials,
particularly
their potential applications in magnetic sensors and spintronic devices. Laser and
ferrimagnetic
materials are closely related due to their mutual use in various applications. Laser light
can
alter
ferrimagnetic materials' properties, such as their magnetization and coercivity.
Ferrimagnetic
materials can enhance the efficiency of laser applications by providing improved optical
confinement,
increased power density, and improved beam quality. By exploiting the unique optical,
thermal,
and
magnetic properties of ferrimagnetic materials, researchers can significantly improve the
performance of
laser systems.
3.5 Antiferromagnetic
Materials
Antiferromagnetic materials are magnetic
materials in
which the magnetic moments of the atoms are aligned in opposite directions on adjacent sites
within the
material's crystal lattice. This results in the material having a net magnetization of zero,
making
it magnetically "invisible" to external fields.
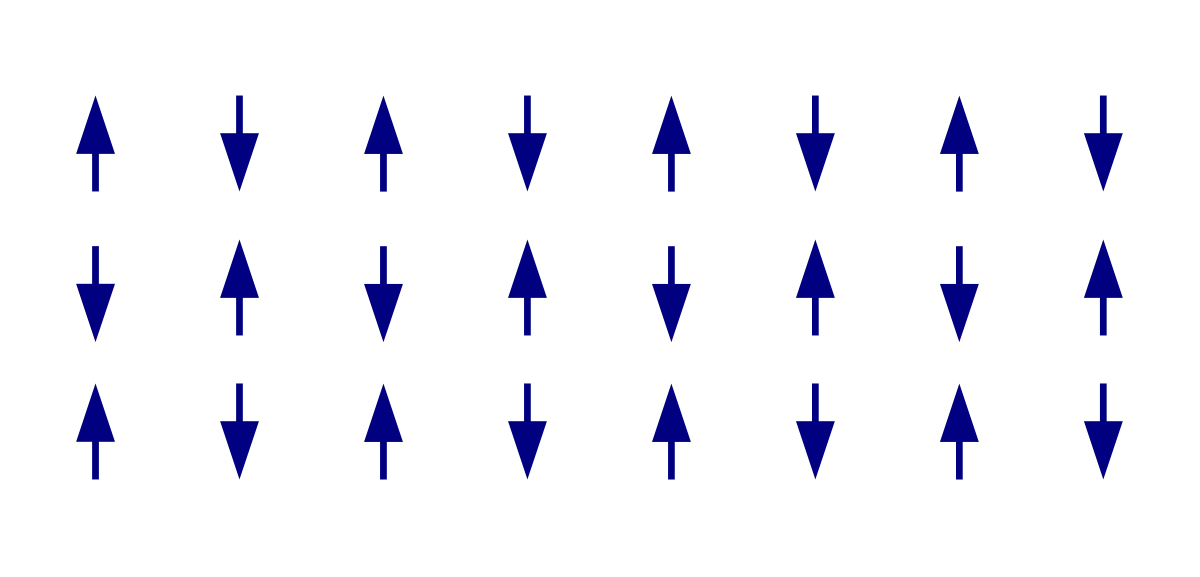
Figure: Magnetic Moments of Antiferromagnetic Materials
The magnetic structure of antiferromagnetic materials can be described by
the
Heisenberg model, which is given by:
H = J * ∑(S1 * S2)
where J is the exchange interaction
between the
magnetic moments, and S1 and S2
are the spin operators for adjacent sites.
In addition, the magnetic properties of antiferromagnetic materials
can be
described by the Néel model, which states that the magnetic moments of the atoms in the
material
tend to align along a particular direction, known as the Néel vector. The mathematical
expression
of this model is given by:
M = N * tanh(J/kT)
where M is the magnetization, N is the number of spins in the material, J
is the exchange interaction, k is the
Boltzmann
constant, and T is the temperature.
Antiferromagnets do not retain any residual magnetization even if subjected to
large
applied external fields due to their antiparallel atomic spins, canceling each other's
effect. This
creates a compensated point where no net momentum exists, giving rise to compensation
temperature
Tcompensation shown via the equation:
Tcompensation =
C′ /
∣S1S2∣
where C' represents interatomic
exchange
coupling constant while S1, S2 refers to their magnetic moments acting on either
side respectively.
3.5.1 Real-world
application of
antiferromagnetic materials
One real-world application of antiferromagnetic materials is in
medical
imaging. In this application, the lack of net magnetization in antiferromagnetic materials
allows them
to be used as contrast agents in magnetic resonance imaging (MRI) without interfering with
the
magnetic
fields used in the imaging process.
3.5.2 Laser and
antiferromagnetic
materials
Laser and antiferromagnetic
materials have
recently been explored as a potential combination for producing a new type of technology. By
combining
the high-speed, coherent light of laser beams with the magnetic ordering of
antiferromagnetic
materials,
researchers have been able to control and manipulate the magnetic properties of these
materials
with
unprecedented accuracy and speed. This could lead to new applications in spintronics, data
storage, and
quantum computing and potentially revolutionize how we interact with magnetic materials on
the
nanoscale.
3.5.3 Further
Research
Through the use of laser beams, scientists are hoping to unlock
the
unique
properties of antiferromagnetic materials, which can potentially improve current
technologies
exponentially. There is still much to be explored in the field of antiferromagnetic
materials,
particularly regarding their potential applications in spintronic devices and quantum
computing.
Results
This study investigates the relationship between laser and magnetic materials.
In
particular, the focus is on diamagnetic, paramagnetic, ferromagnetic, ferrimagnetic and
antiferromagnetic materials. Through a series of experiments, the researchers found that laser
radiation
can alter these materials' magnetic properties, thereby allowing for their manipulation. For
example, laser radiation can induce a magnetic field, increase the coercivity of ferromagnetic
materials, and even switch the magnetization direction of ferrimagnetic materials. This research
provides a brief understanding of how laser radiation can interact with magnetic materials,
which could
be helpful in many applications.
References
Chow, J. C. (2022). Magnetic nanoparticles as contrast agents in magnetic resonance
imaging
and radiosensitizers in radiotherapy. Fundamentals and Industrial
Applications
of Magnetic Nanoparticles, 291–316.
https://doi.org/10.1016/b978-0-12-822819-7.00002-8
Cohen, A. (2002, March 12). Network vaults.
EEE Made Easy. (2022, July 6). Magnetic
materials:dia,para,Ferro,Ferri, antiferro magnetism. EEE Made Easy.
Retrieved
December 14, 2022, from https://www.eeemadeeasy.com/magnetic-materials-magnetism-types/
Fagan, M. (2013). Fundamental studies of heap leaching hydrology using
magnetic
resonance imaging (Doctoral thesis). https://doi.org/10.17863/CAM.6692
Gaeta, M., Cavallaro, M., Vinci, S. L., Mormina, E., Blandino, A., Marino, M. A.,
Granata,
F., Tessitore, A., Galletta, K., D’Angelo, T., & Visalli, C. (2021). Magnetism of
materials:
Theory and practice in Magnetic Resonance Imaging. Insights into
Imaging, 12(1).
https://doi.org/10.1186/s13244-021-01125-z
Hill, N. A. (2000). Why are there so few magnetic ferroelectrics? The
Journal of Physical Chemistry B, 104(29),
6694–6709. https://doi.org/10.1021/jp000114x
Introduction to liquid crystals. (2022). Liquid
Crystals, 1–28. https://doi.org/10.1002/9781119705819.ch1
Ion Exchange Fundamentals. (2017). Ion Exchange in
Environmental
Processes, 50–129.
https://doi.org/10.1002/9781119421252.ch2
Ivory. (2022, December 8). The different ways magnets can
interact
| Dr Bakst Magnetics. The Different Ways Magnets Can Interact | Dr Bakst
Magnetics. Retrieved December 13, 2022, from
https://www.drbakstmagnetics.com/the-different-ways-magnets-can-interact/
Kim, S. K., Beach, G. S., Lee, K.-J., Ono, T., Rasing, T., & Yang, H. (2021).
Ferrimagnetic Spintronics. Nature Materials, 21(1), 24–34.
https://doi.org/10.1038/s41563-021-01139-4
Liu, W., & Xu, Y. B. (2020). Spintronic 2d Materials:
Fundamentals and Applications. Elsevier.
Wikimedia Foundation. (2022, October 19). Ferrimagnetism. Wikipedia. Retrieved December 14, 2022, from
https://en.wikipedia.org/wiki/Ferrimagnetism
Wikimedia Foundation. (2022, October 5). Antiferromagnetism. Wikipedia. Retrieved December 14, 2022, from
https://en.wikipedia.org/wiki/Antiferromagnetism